Gamma-ray Astrophysics
Involving Research Members
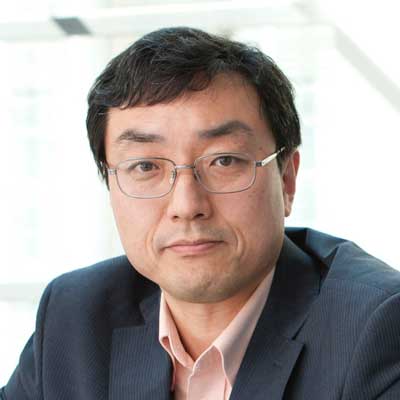
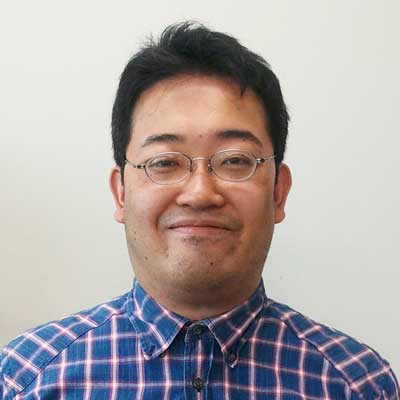
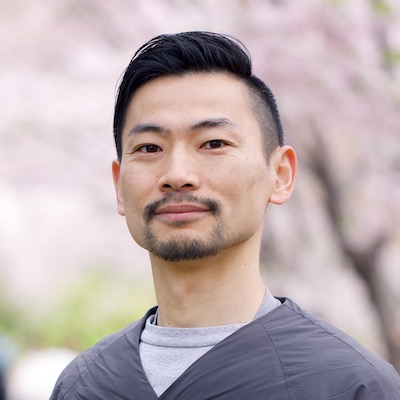
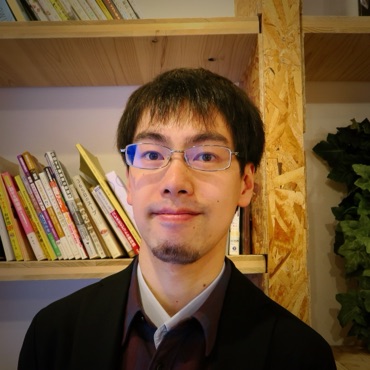
- Gamma-ray Observation for Cosmic Ray Research
- The Origin of Cosmic Rays Revealed by the Fermi Gamma-ray Space Telescope (Fermi Satellite)
- Study of Gamma-Ray Sources With the Fermi Satellite
- New X-ray Satellite: Hitomi (ASTRO-H)
- Further Development of Gamma-ray Observation
- The Origin of Extragalactic Cosmic Rays
Gamma-ray Observation for Cosmic Ray Research
Cosmic rays are high-energy radiation that come down from space and have a wide energy range from at least 109 electron volts to more than 1020 electron volts. Cosmic rays were discovered by the Austrian scientist Victor Franz Hess more than 100 years ago in 1912. At the time, it was thought that the spontaneous discharge of the electroscope was caused by radiation from the Earth’s crust. When Hess and his colleagues measured the altitude dependence of radiation using a balloon, they discovered that the radiation level is hugher at higher altitude. With this discovery, it became clear that high-energy radiation is coming from outside the earth, i.e., from outer space, and was called cosmic rays.
Since the energy of cosmic rays far exceeds what humans can achieve with accelerators, the physical processes involved in their acceleration are a very interesting mystery. The first step in understanding the physical process of cosmic ray production is to find the origins of the cosmic rays. Unfortunately, cosmic rays, which are charged particles, cannot travel straight due to the turbulent interstellar magnetic field, so measuring their arrival direction will not lead us to their origins. Gamma rays emitted by the interaction between cosmic rays and interstellar gas can travel straight without being affected by the interstellar magnetic field, and are considered to be the most promising method to study the origin and propagation of cosmic rays. The gamma-ray group at CR-lab is working on the gamma-ray observation described below, and conducts cosmic-ray research by observing gamma rays over a nine-decades of energyband from 100 keV (105 electron volts) to 100 TeV (1014 electron volts).
The Origin of Cosmic Rays Revealed by the Fermi Gamma-ray Space Telescope (Fermi Satellite)
The Fermi satellite (see Figure 1) has detected more than 1,800 gamma-ray sources since its launch in 2008, and has brought major progress in gamma-ray astrophysics. In the study of cosmic-ray origin, the Fermi satellite observations have provided conclusive evidence. Supernova remnants were considered to be the most promising candidate for the origin of cosmic rays in our galaxy. The Fermi satellite observed gamma rays from relatively old supernova remnants (more than tens of thousands of years after the explosion) called W44, W51C and IC443. In these supernova remnants, there were indications that the shock waves were interacting with the surrounding interstellar gas, so it was expected that gamma rays originating from cosmic-ray protons could be observed. However, as shown in Fig. 2, our image restoration method revealed that in W44 and IC443, the gamma rays are emitted from the shock wave region of the supernova remnant.
In addition, the energy spectra of W44 and IC443 below 200 MeV (2 × 108 electron volts) were precisely measured, and the spectral characteristic of gamma rays originating from cosmic-ray protons were successfully observed as shown in Fig. 3. When cosmic-ray protons interact with interstellar gas, they produce neutral pions, which decay into gamma rays. Due to kinematic constraints on the neutral pion production, the production probability of neutral pions increases rapidly with increasing pion energy. The gamma-ray spectrum derived from the electron interaction is much more gradual and cannot explain the observed spectrum. The results of this measurement are conclusive that cosmic-ray protons are being accelerated in supernova remnants.
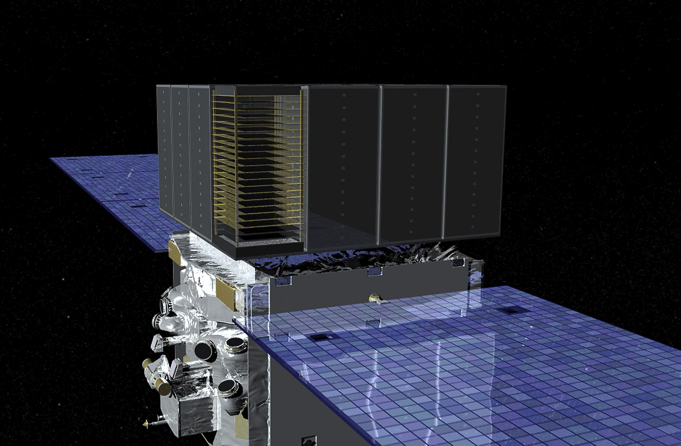
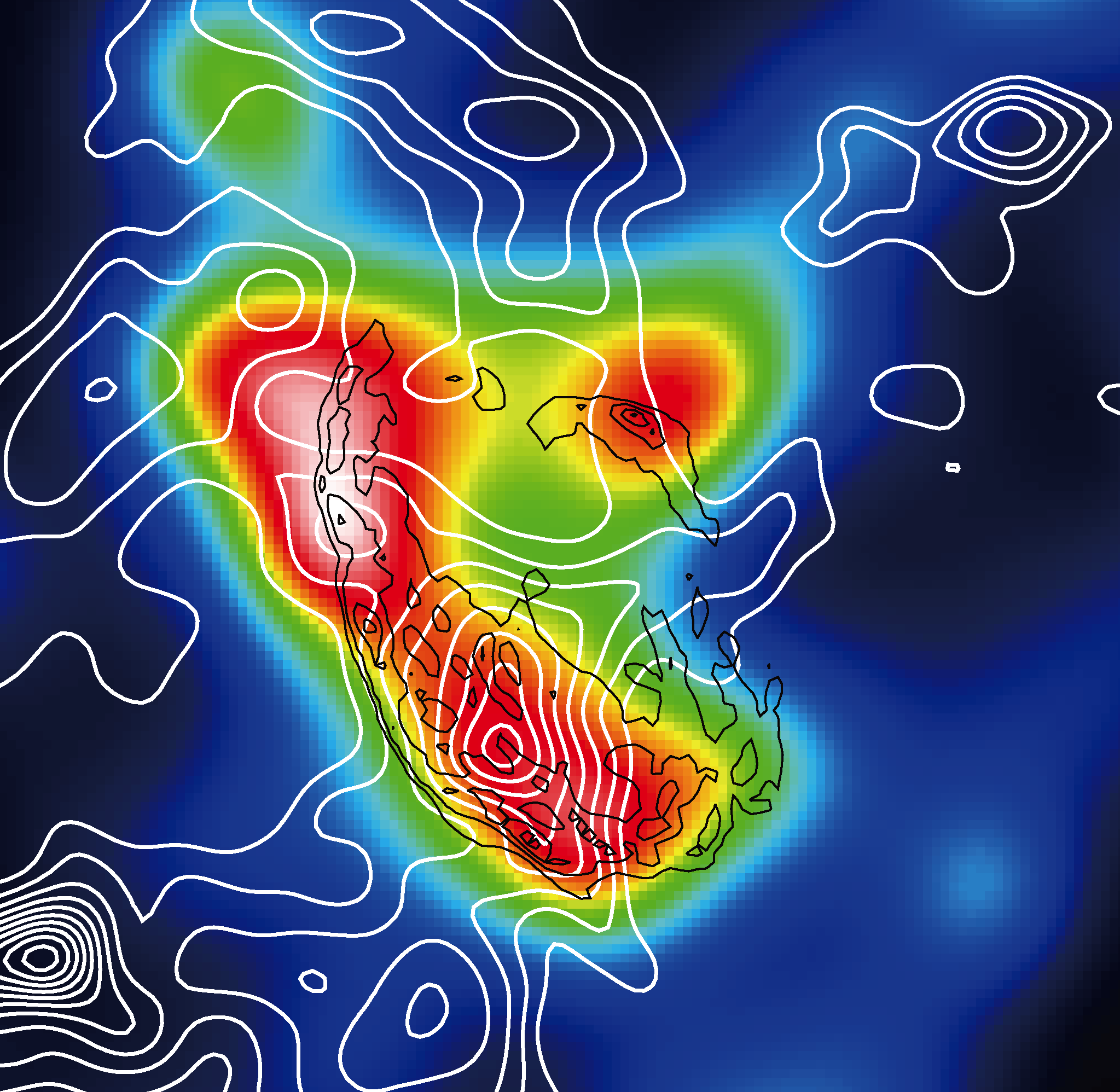
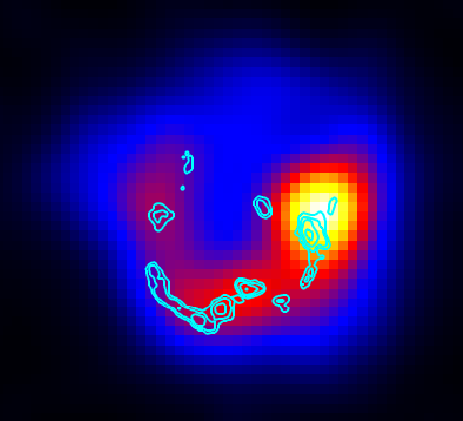
The color map shows the intensity of gamma rays, the black contours on the left show the intensity of radio waves from synchrotron radiation of electrons accelerated in the shock wave, the white contours show the interstellar-gas density, and the light blue contours on the right show the density distribution of the interstellar gas heated by the shock wave. This observation revealed that gamma rays are emitted where shock waves from supernova explosions collide with the interstellar gas. Our group is also working on image analysis of other supernova remnants.
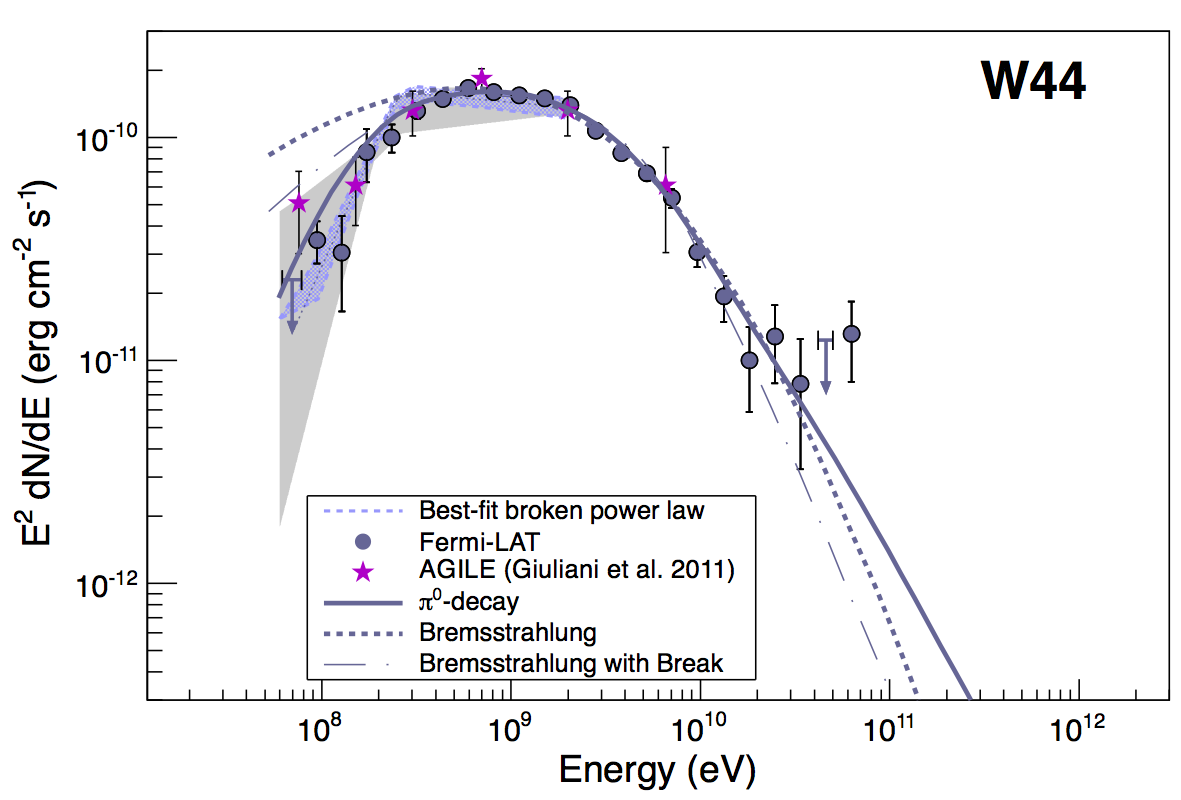
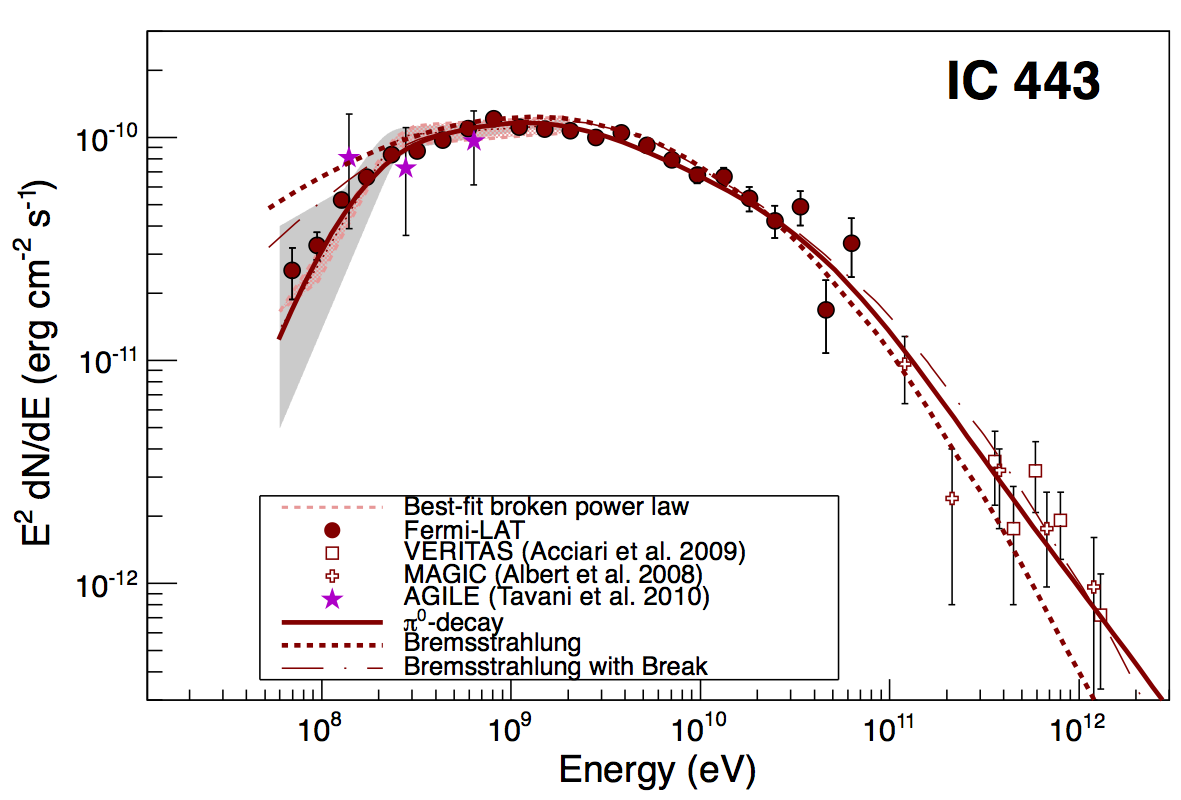
The spectra observed by the Fermi satellite are in good agreement with those expected for a proton origin, whereas they do not match those expected from electron bremsstrahlung.
Study of Gamma-Ray Sources With the Fermi Satellite
In addition to supernova remnants, the Fermi satellite has detected more than 1000 active galactic nuclei, providing new experimental insights into the spatial distribution and temporal evolution of each type, and greatly advancing our understanding of the process of galaxy formation. The Fermi satellite has detected more than 120 pulsars, nearly half of which were detected for the first time, and has made great progress in the study of pulsars. In particular, the Fermi satellite’s observations have led to the detection of a larger number of binary pulsars called millisecond pulsars than previously thought, which has become a hot topic.
In addition, the Fermi satellite has made an important contribution to the search for dark matter. There has been a lot of talk about the increase in the spectrum of cosmic ray electrons, which may have originated from dark matter, as measured by the Cosmic-Ray Balloon Experiment ATIC. However, precise spectral measurements by the Fermi satellite ruled this out. On the other hand, the gamma-ray signal produced by the annihilation of dark matter is also a promising approach to detect dark matter. The Fermi satellite searched for dark matter with the highest sensitivity using five years of gamma-ray data, but found no evidence of it. The results showed that there is no evidence of dark matter below a mass of 100 GeV (1011 electron volts, about 100 times the mass of a proton), placing a strong constraint on the mass range of dark matter. With further observations, we expect to be able to detect dark matter masses below 800 GeV. Our group is studying the detailed distribution of gamma-ray emission around the center of the galaxy, which is thought to have the highest density of dark matter in our vicinity, and is working to detect the gamma-ray signal emitted by dark matter annihilation.
New X-ray Satellite: Hitomi (ASTRO-H)
The Hitomi satellite (see Figure 4) is JAXA’s X-ray satellite launched on February 7, 2016. The Hitomi satellite is capable of X-ray spectroscopy (measuring the energy spectrum) with excellent energy resolution (less than 7 electron volts), imaging spectroscopy (taking images and measuring the energy spectrum at the same time) up to the hard X-ray region (0.3 to 80 keV), and X-ray and gamma-ray spectroscopy in a wide energy band (0.3 to 600 keV). It also features X-ray and gamma-ray spectroscopy in a wide energy band (0.3 to 600 keV). To realize high-resolution and broadband observations, the Hitomi satellite is equipped with four types of instruments. Among them, the Soft Gamma-ray Detector (SGD), which our group is leading the development of, provides 10 times higher sensitivity in the soft gamma-ray region from 40 keV to 600 keV. The SGD combines a semiconductor Compton camera, which can measure the direction and energy of gamma-rays with good resolution, and a collimator, which restricts the direction of gamma-rays, to eliminate background gamma-rays from outside of the filed of view and achieve unprecedented sensitivity. Our group contributed to the development of silicon semiconductor detectors and dedicated integrated circuits.
The Hitomi satellite had to cease operations due to an attitude control system failure that occurred soon after launch. Our group is now analyzing the data of the celestial objects that we were able to observe with SGD before that.
The semiconductor Compton camera developed to observe faint gamma rays from space is capable of identifying gamma rays specific to radioactive materials such as cesium, and also has the ability to visualize widely distributed radioactive materials in a wide field of view. With the help of a grant from the Japan Science and Technology Agency, we are working to commercialize a portable camera that can be used outdoors, and to utilize this technology for the decontamination of radioactive materials in Fukushima to help with reconstruction efforts. (See press release)
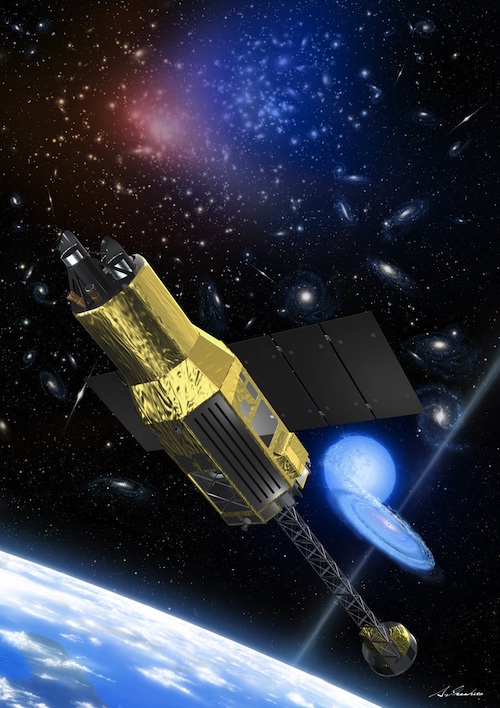
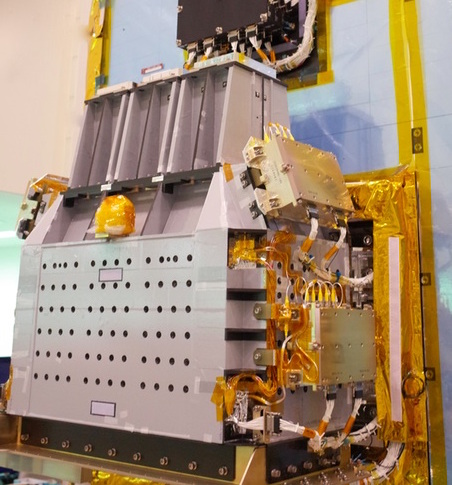
Further Development of Gamma-ray Observation
Previous observations with the Fermi satellite revealed that cosmic rays are accelerated in supernova remnants up to about 1 TeV (1012 electron volts). However, since the spectrum of cosmic rays changes around 1015 to 1016 electron volts, it is thought that cosmic rays are accelerated in supernova remnants at least up to that energy range, but direct evidence for this has not yet been obtained. In order to observe gamma-rays due to cosmic ray interactions at energies higher than those of the Fermi satellite, it is essential to develop instruments with sufficient sensitivity in higher energy bands. The next generation atmospheric Cherenkov telescope project, [Cherenkov Telescope Array (CTA)] (http://www.cta-observatory.org/), is currently under development, and will consist of nearly 100 telescopes of three different sizes, as shown in Figure 5. The CTA is being developed to extend the lower and upper limits of the observed energy by an order of magnitude compared to the previous atmospheric Cherenkov telescopes, and to improve the detection sensitivity of gamma-ray sources by a factor of 10. Large aperture telescopes can detect gamma-rays with lower energies due to their high light collecting capability, while small aperture telescopes can only detect gamma-rays with higher energies, but the cost per telescope can be greatly reduced, making it possible to install dozens of telescopes. Since the flux of high-energy gamma rays decreases in inverse proportion to the second or third power of the energy, the increase in the number of telescopes will directly lead to an increase in the detection sensitivity in the high-energy region, which in turn will contribute to increasing the upper limit of the observation energy band by one order of magnitude. Thus, by appropriately mixing telescopes of different sizes according to the observation energy range, we can achieve broadband and high-sensitivity observations. As a result, the CTA will be able to observe all supernova remnants in the Galaxy, whereas previously it could only observe supernova remnants as far as the Galactic center. Therefore, it is expected that the CTA will be able to observe more supernova remnants at higher energies, which will further advance the study of cosmic-ray acceleration. In addition, the angular resolution will be improved by a factor of about three, which will enable us to observe the gamma-ray morphology of supernova remnants in more detail, which will be useful for studying the gamma-ray emission mechanism.
The CTA consists of four large-sized telescopes (about 23 m in diameter), two dozen medium-sized telescopes (about 12 m in diameter), and several dozen small-sized telescopes (about 4 m in diameter).
At CTA, we are vigorously pursuing development to realize the instruments. Our group is working on the development of a semiconductor photon sensor, which is the most promising new technology. Conventional Cherenkov telescopes use photomultiplier tubes to efficiently capture the dozens of Cherenkov photons per square meter generated by the interaction of gamma rays with the atmosphere. Recently developed semiconductor photon sensors (Silicon Photomultiplier, SiPM) utilizing Geiger-mode avalanche photodiodes have the same level of photoelectron amplification as photomultiplier tubes, but with about twice the photon-detection efficiency. In addition, SiPMs have high expectations as photon sensors because they can reduce the cost per pixel and have high durability. On the other hand, there are some drawbacks, such as crosstalk into neighboring cells during the photomultiplying process, so development to improve performance is being vigorously pursued. In November 2015, a prototype of the small-sized telescope equipped with the integrated circuit developed by our group was completed, and it was the first CTA prototype to successfully observe Cherenkov light from cosmic rays (see Figure 6).
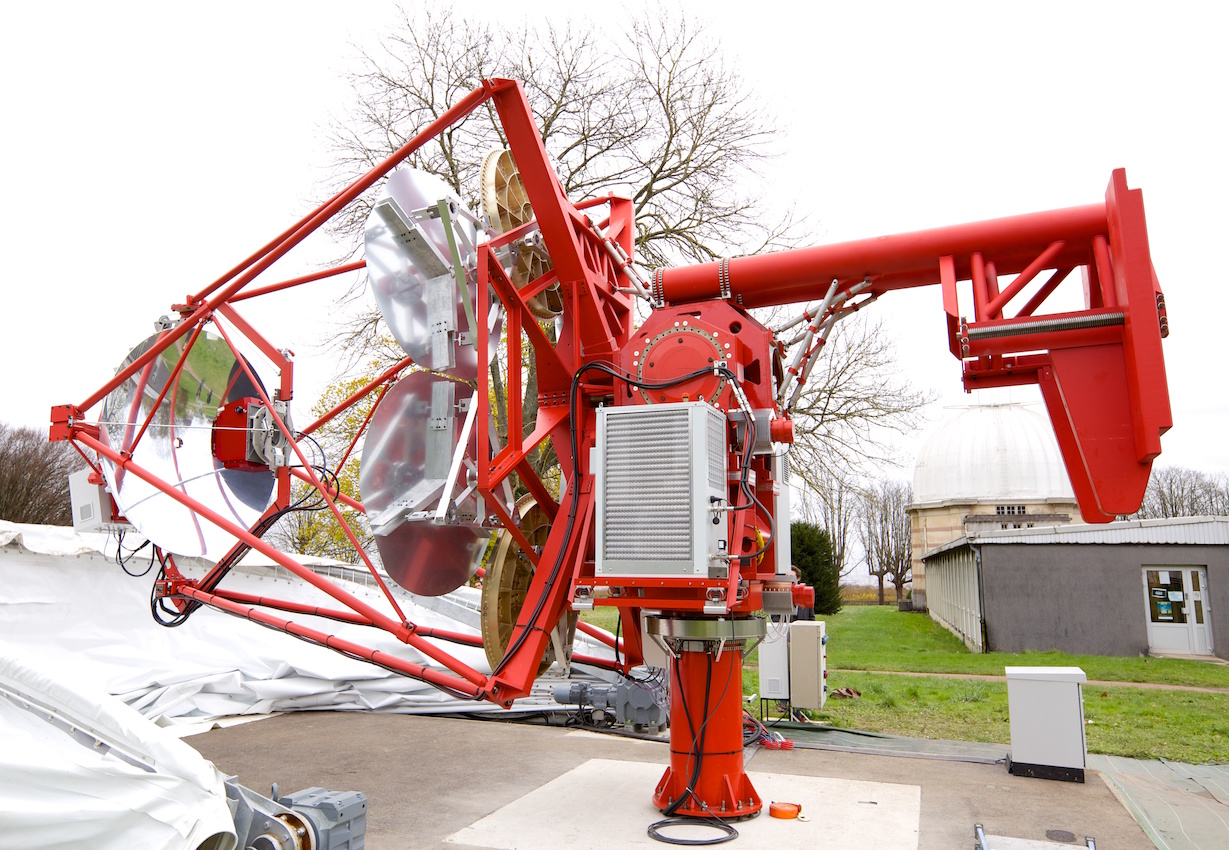
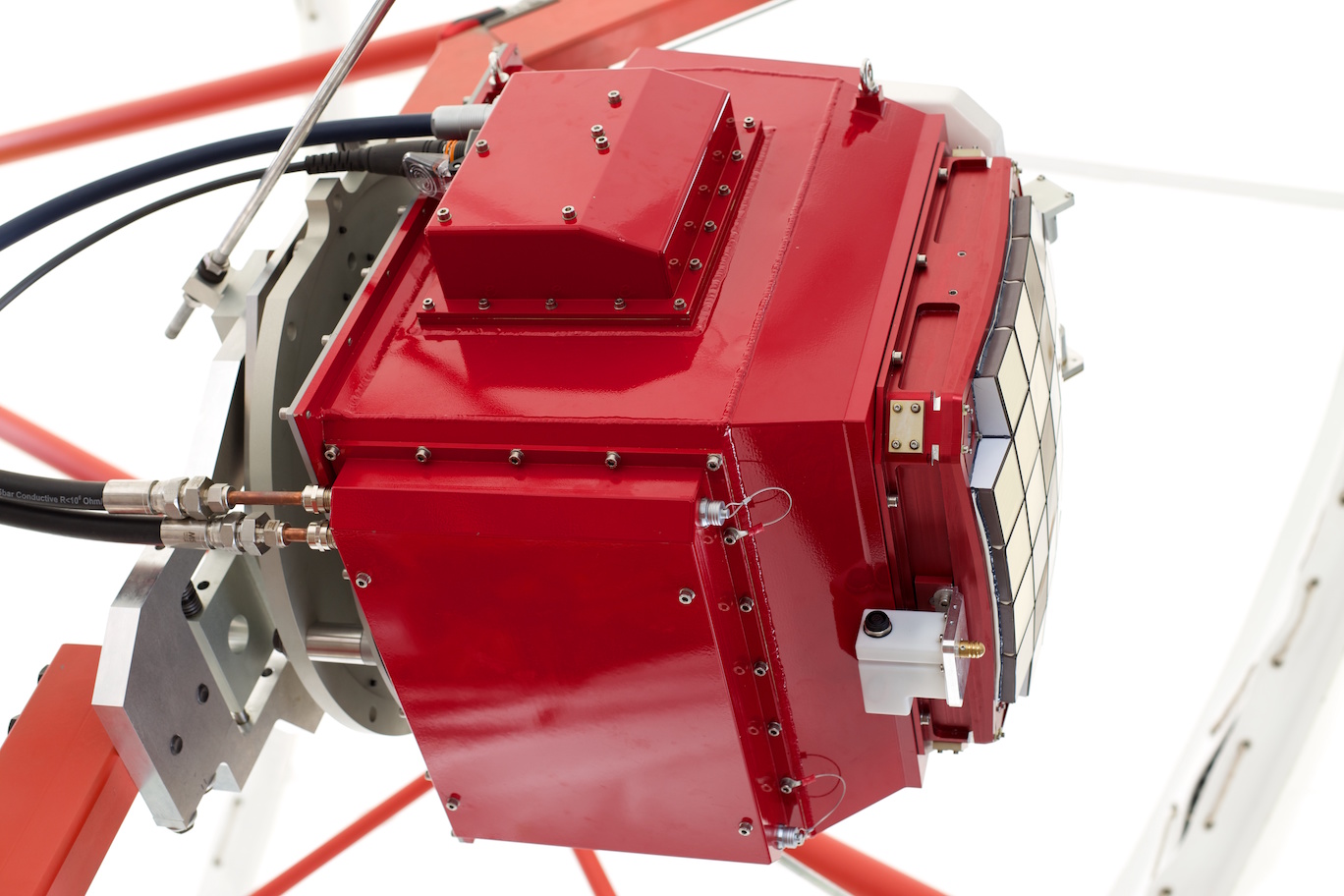
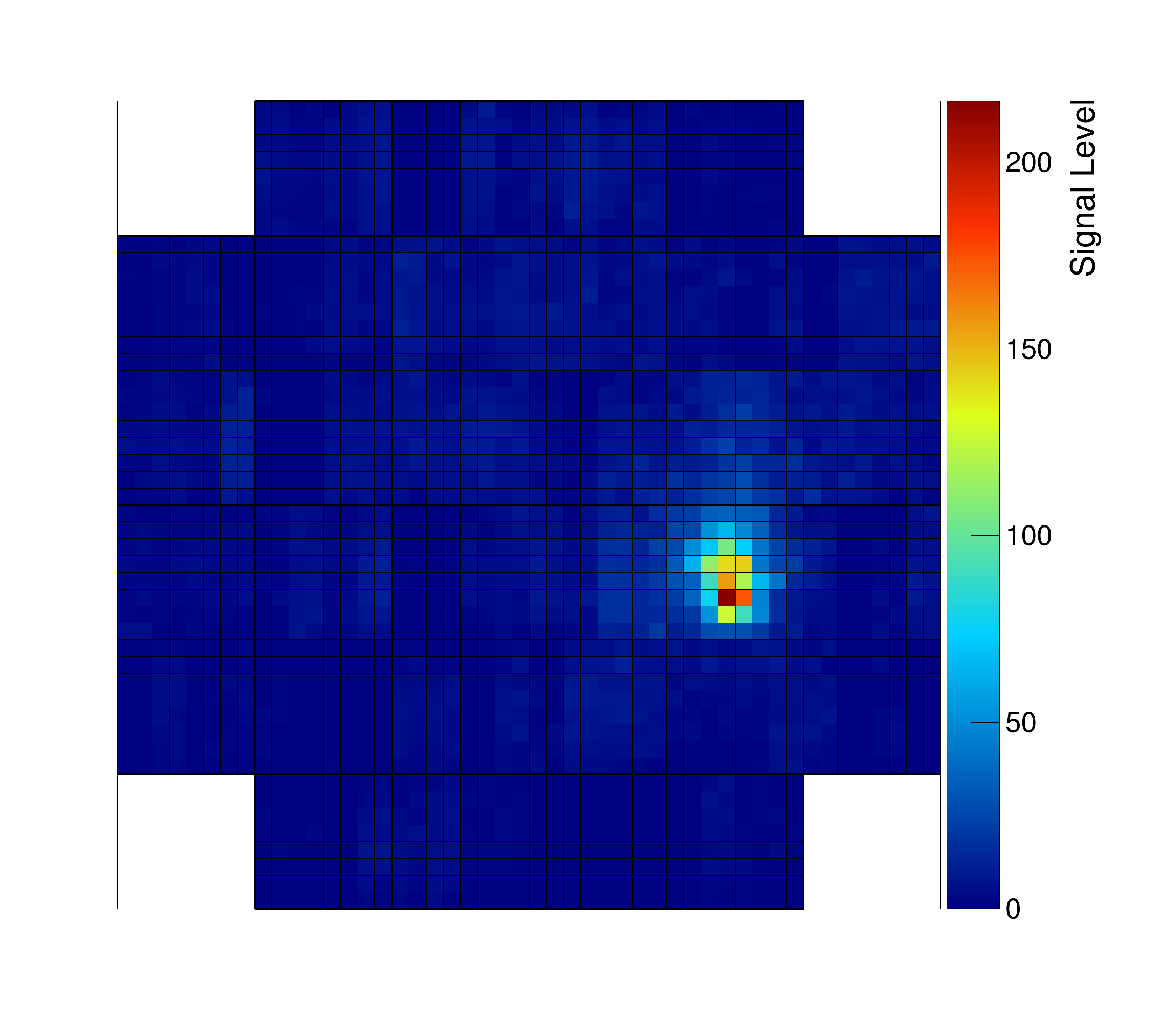
The Origin of Extragalactic Cosmic Rays
So far, we discussed cosmic-ray origins within our galaxy. Considering the strength of the interstellar magnetic field in the Milky Way, cosmic rays with energies above 1017 eV cannot stay in the Milky Way. It is believed that most of the cosmic rays above 1017 eV come from extragalactic sources. There are several theories about the origin of extragalactic cosmic rays, such as “hypernovae” explosions that cause gamma-ray bursts, supermassive black holes with masses one million to one billion times that of the sun, and turbulence caused by the collision and subsequent merging of galaxy clusters. High-energy gamma-ray observations by the CTA are expected to reveal extragalactic cosmic ray sources. For example, Fermi satellite observations have shown that high-energy gamma rays are emitted with a delay of more than 10 seconds in gamma-ray bursts. This phenomenon is thought to indicate the proton origin, but due to the insufficient sensitivity of the Fermi satellite, the number of gamma-rays that can be observed is limited, and the radiation mechanism has not yet been understood. For such transient phenomena, the CTA is 100,000 times more sensitive than the Fermi satellite (in the energy region of several tens of GeV), and is expected to clarify the gamma-ray radiation mechanism in gamma-ray bursts by detecting a large number of gamma rays. However, the CTA’s field of view is much narrower than that of the Fermi satellite, so its observation rate is estimated to be about once a year. Gamma rays from supermassive black holes at redshifts of 0.1 or more are thought to interact with the background infrared light and attenuate, but there are recent reports that contradict this expectation. Some believe that the gamma rays from these supermassive black holes can be explained by a cascade of interaction between the cosmic miscrowave background and the ultra-high-energy cosmic-ray protons accelerated by the supermassive black holes.