Neutrino Studies
Involving Research Members
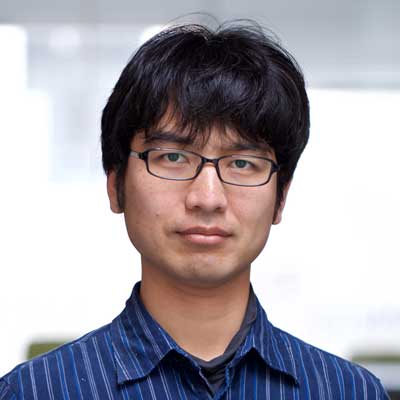
Neutrino Studies
Neutrino Oscillations
Neutrinos are subatomic particles with little mass, neutral, which merely interact with material because they feel only weak interactions. Corresponding to the three types of charged leptons: electron, muon, and tau, there are three types of neutrinos: electron neutrinos, muon neutrinos, and tau neutrinos, which exchange charge with their corresponding charged lepton counterparts by the weak interaction. While there are three neutrino masses, m1, m2, and m3, a mass of muon neutrino, for example, is not simply m2, but a combination of the quantum states of m1, m2, and m3. This is so-called neutrino mixing (Fig. 1). Because of neutrino mixing, neutrinos undergo neutrino oscillations, in which their type changes during flight (and the probability of being detected by that type changes) (Fig. 2). From the measurement of neutrino oscillations, we can explore the neutrino mixing and neutrino masses. Professor Takaaki Kajita (current director of the Institute for Cosmic Ray Research, the University of Tokyo) discovered atmospheric neutrino oscillations at Super-Kamiokande in 1998, and at the same time, this meant the first evidence that neutrinos have finite mass. For these achievements, Prof. Kajita was awarded the 2015 Nobel Prize in Physics. In 2001, Super-Kamiokande and the SNO experiment showed that the solar neutrino deficit since 1970 was also due to neutrino oscillations, followed by the KamLAND experiment, which found anti-electron neutrino oscillations from nuclear reactors, and the K2K experiment, which found accelerator muon neutrino oscillations. In 2012, all the three mixing angles of neutrinos were finally measured by T2K and Daya Bay, etc. In 2020, T2K provided the results suggesting a difference in the behavior of particles and antiparticles in muon-neutrino oscillations into electron-neutrinos (CP violation in the neutrino sector ). This may give a hint of the reason why our universe is made of only matter and the anti-universe does not seem to exist (matter-antimatter asymmetry).
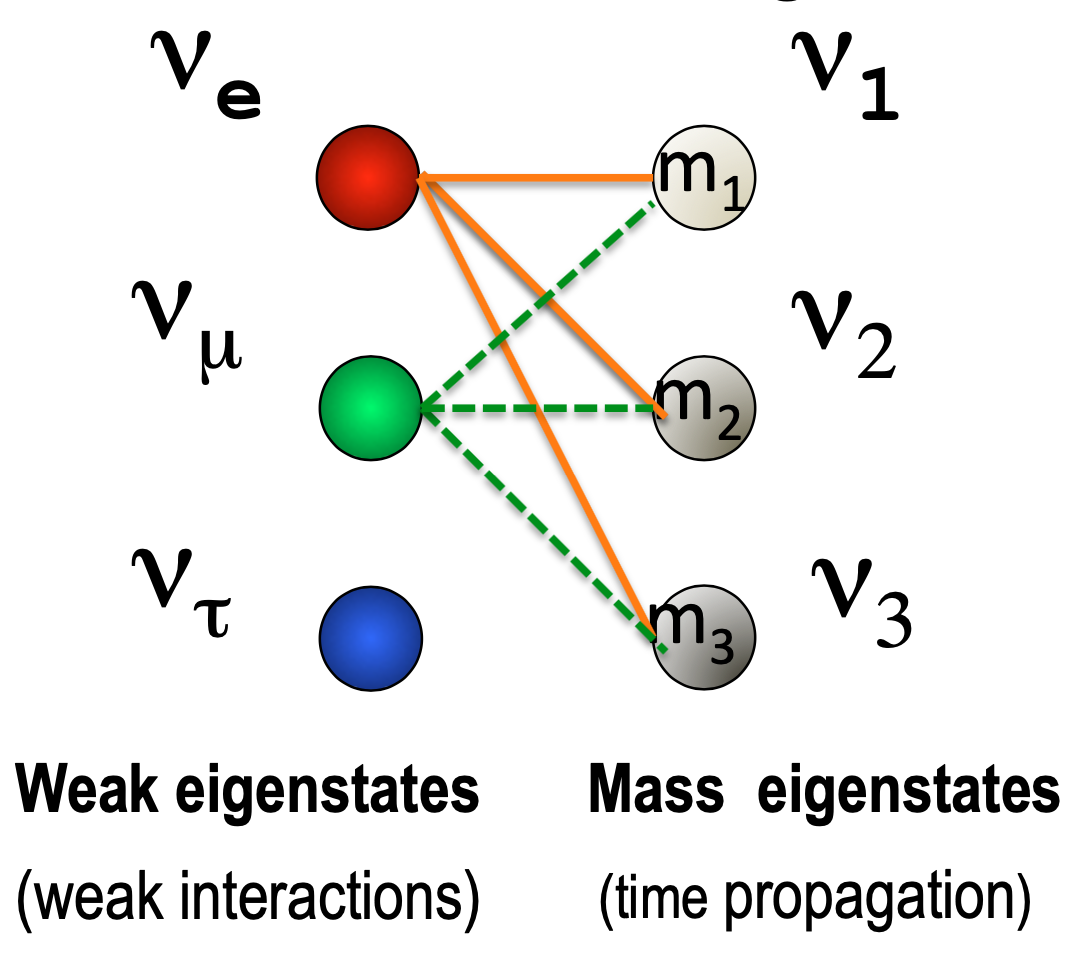
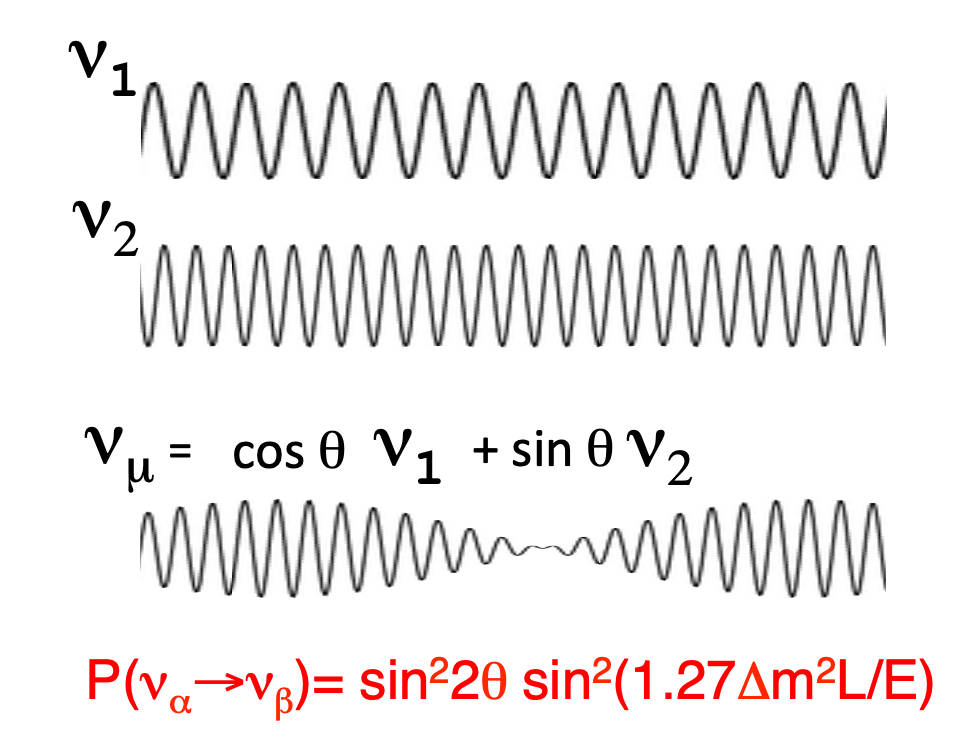
Proton Decay
Another important goal of Super-Kamiokande is to discover proton decay. Proton decay is a phenomenon in which a proton, which is considered to be inherently stable, decays into a combination of other lighter particles. Since the proton is the lightest particle with a baryon number of one, and the lighter particles are mesons, leptons, and other particles that do not have a baryon number, the observation of proton decay means that the baryon number is not conserved. Reactions that do not conserve the baryon number are one of the conditions under which matter-antimatter asymmetry occurs in the early universe (Sakharov’s three conditions). Super-Kamiokande has been searching for events where protons decay into neutral pions and positrons, and charged K mesons and neutrinos, and so on, but has yet to find any.
Neutrino Astronomy
Neutrinos, which have no electric charge and thus travel straight through space, are expected to become the new eyes for observing the universe, in addition to electromagnetic waves (visible light, radio waves, and gamma rays). Only two astronomical objects in which neutrinos have been detected so far are the Sun, where neutrinos associated with thermonuclear fusion have been detected, and SN 1987A, a core-collapse supernova explosion that occurred in the Large Magellanic Cloud in 1987. The Kamiokande experiment, the predecessor to the Super-Kamiokande experiment, firstly detected the burst of neutrinos from SN 1987A and won Prof. Masatoshi Koshiba the Nobel Prize in Physics in 2002. In recent years, neutrino astronomy has made great progress with the detection of high-energy neutrinos from celestial objects by IceCube, and neutrinos from the Blaser object TXS 0506+056. The Super-Kamiokande experiment is also continuing its research on the search for neutrinos from supernova explosions and neutrinos from transient objects such as gravitational waves (see below). The search for neutrinos from celestial objects is also being used for particle physics research, such as an indirect search of dark matter through the observation of high-energy neutrinos from the Sun or the galactic center.
Super-Kamiokande Experiment
Super-Kamiokande is a neutrino detector that stores 50,000 tons of ultra-pure water in a cavity 40 m in diameter and 40 m high, 1000 m underground in the Kamioka mine (Fig. 3). Cherenkov light from the particles produced by the interaction of neutrinos with water is observed using about 11,000 of 50 cm diameter photomultiplier tubes equipped to the walls of the cavity. Since the Cherenkov light is emitted in a ring in the direction of the particles, the position, direction, type, and the number of particles can be reconstructed from the pattern of the light (Fig. 4). 1000 m of bedrock stops most cosmic rays from outside, and the ultrapure water itself blocks radiation from the bedrock, so the center of the tank is free of any incoming particles and is ideal for studying rare events such as neutrino reactions and proton decay. Super-Kamiokande has been at the forefront of neutrino research for almost 25 years since it started observations in April 1996. In summer 2020, SK-Gd, which will increase neutron detection capability by dissolving gadolinium in water, will start (see below). At CR Lab, Prof. Itow has been one of the founding members of Super-Kamiokande since 1995 and leading the experiment with a major role in the discovery of neutrino oscillations in atmospheric and accelerator neutrinos.
Super-Kamiokande Experiment official web site
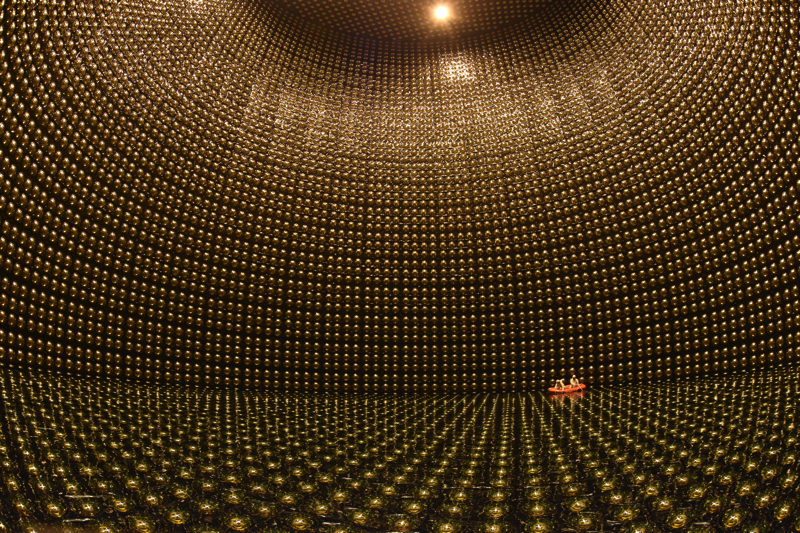
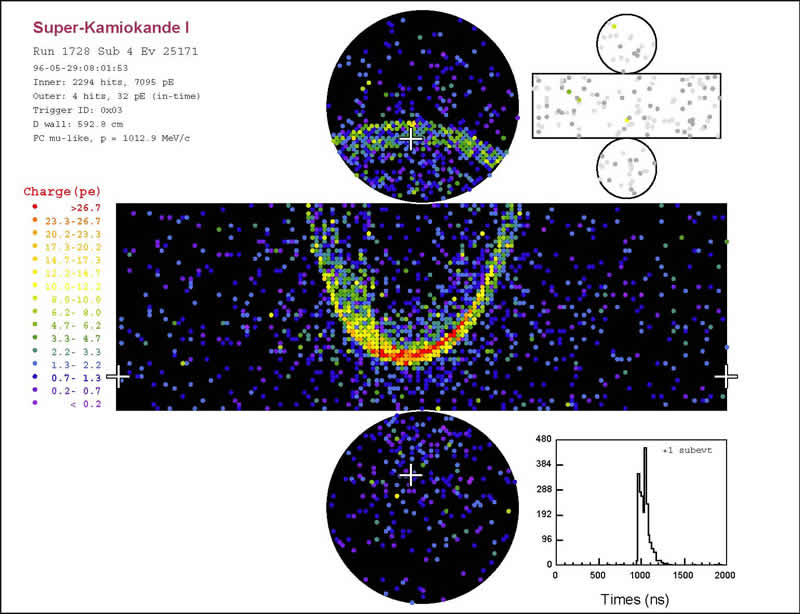
Neutrino Research at CR Lab
CR Lab conducts a variety of neutrino research using Super-Kamiokande. The following is a list of typical activities. In addition to these, we are also involved in hardware activities, such as PMT development for the Hyper-Kamiokande experiment (see below).
-
Precise Observation of Atmospheric Neutrino Oscillations
Atmospheric neutrinos are produced in the air-showers which are created by cosmic rays colliding with the Earth’s atmosphere. There produced muon neutrinos and electron neutrinos mainly from the decay of charged pions and kaons. Atmospheric neutrinos have energies spreading over a wide range from MeV to PeV, and can penetrate the Earth even from the far side of the Earth to be detected by Super-Kamiokande, making it possible to detect the matter effect in neutrino oscillations through the matter of Earth. This makes Super-Kamiokande ideal for studying the ordering of the three neutrino mass levels (mass hierarchy), and for investigating the possible unknown interactions of neutrinos with the matter. For this purpose, it is necessary to analyze neutrinos and antineutrinos separately. Our group is developing methods for analyzing data from Super-Kamiokande while searching for non-standard interactions of neutrinos and exploring the mass hierarchy through precise analysis of atmospheric neutrinos. -
Atmospheric Neutrino Modeling
To study atmospheric neutrino oscillations, it is necessary to accurately predict the energy, distance, and flux of neutrinos that are measured by the SK detector. We are currently working on improving the accuracy of modeling atmospheric neutrino production for more sensitive oscillation measurements. Cosmic ray air showers develop through repeated “hadronic interactions” in which high-energy protons or nuclei collide with the nuclei of the atmosphere. Many accelerator experiments, such as the LHCf/RHICf or various fixed target experiments as NA61, have attempted to precisely measure the hadronic interaction. In our group, we are trying to improve the accuracy and reliability of simulations and to expand the applicable energy range by reflecting these accelerator measurements in our calculations. -
Search for Neutrinos in Dark Matter
When dark matter collides with each other, it is supposed to undergo annihilation and produce ordinary particles such as cosmic rays including neutrinos. If dark matter has a lifetime, it can also decay into neutrinos. Dark matter trapped by the gravitational potential of the Sun, the Earth, or the center of a galaxy may be emitting high-energy neutrinos. We are using the penetrating power of neutrinos to search for dark matter by searching for neutrinos from dark matter that has accumulated in the interiors of celestial bodies. -
Search for Neutrinos from Sudden Objects such as Gravitational Waves
Multi-messenger astronomy, which combines optical observations of celestial objects with other observations, has rapidly progressed since the detection of gravitational waves by LIGO/Virgo. Among gravitational wave astronomical events, neutrino emission is expected in the merger of neutron-star binary and core-collapse supernova explosions, and we are aiming to detect these events with Super-Kamiokande. When a gravitational wave is detected in a gravitational wave observatory, an alert is sent immediately, and a search for neutrino events synchronized with the gravitational wave event is conducted in real-time. In addition to gravitational waves, we are also preparing to search for neutrinos from transient sources such as gamma-ray bursts.
Future Plans
SK-Gd Project
In summer 2020, gadolinium has been dissolved in the pure water of Super-Kamiokande, and it is now possible to efficiently detect anti-electron neutrinos produced by supernova explosions. Gadolinium has an extremely large capture cross-section for neutrons, and when captured, emits gamma rays. By observing both the signal from the neutrino reaction and the delayed signal from the neutrons, we can efficiently identify anti-electron neutrino events (Fig. 4). This research will not only improve our ability to observe supernova explosions in our galaxy but will also help us to understand the mystery of the formation of heavy elements in the universe by observing the integration of neutrinos produced by supernova explosions in the past.
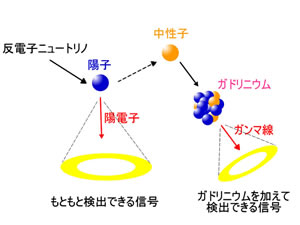
Hyper-Kamiokande Experiment
The Hyper-Kamiokande experiment is the successor to the Super-Kamiokande experiment. Like Super-Kamiokande, it will use a technique to measure Cherenkov light from particles produced when neutrinos interact with water, but the detector will be much larger. The Hyper-Kamiokande detector is 68 m in diameter and 71 m in height, which makes its effective volume about 8 times larger than that of Super-Kamiokande. This means that the number of events that would take 24 years to observe in Super-Kamiokande can be obtained in 3 years in Hyper-Kamiokande. This increase in the number of events is expected to dramatically accelerate neutrino research, including neutrino oscillations and proton decay. The construction budget has been approved in FY2019, and the cavity construction has finally started in 2020. About 20,000 newly developed 20-inch photomultiplier tubes and a few thousand multi-PMT modules will be installed on the inner walls of the detector to detect the faint light of Cherenkov radiation. The experiment is scheduled to start in 2027. We have been intensively involved in the planning of Hyper-Kamiokande, especially working for the development of new photomultiplier tubes and their test.
Hyper-Kamiokande Experiment official web site
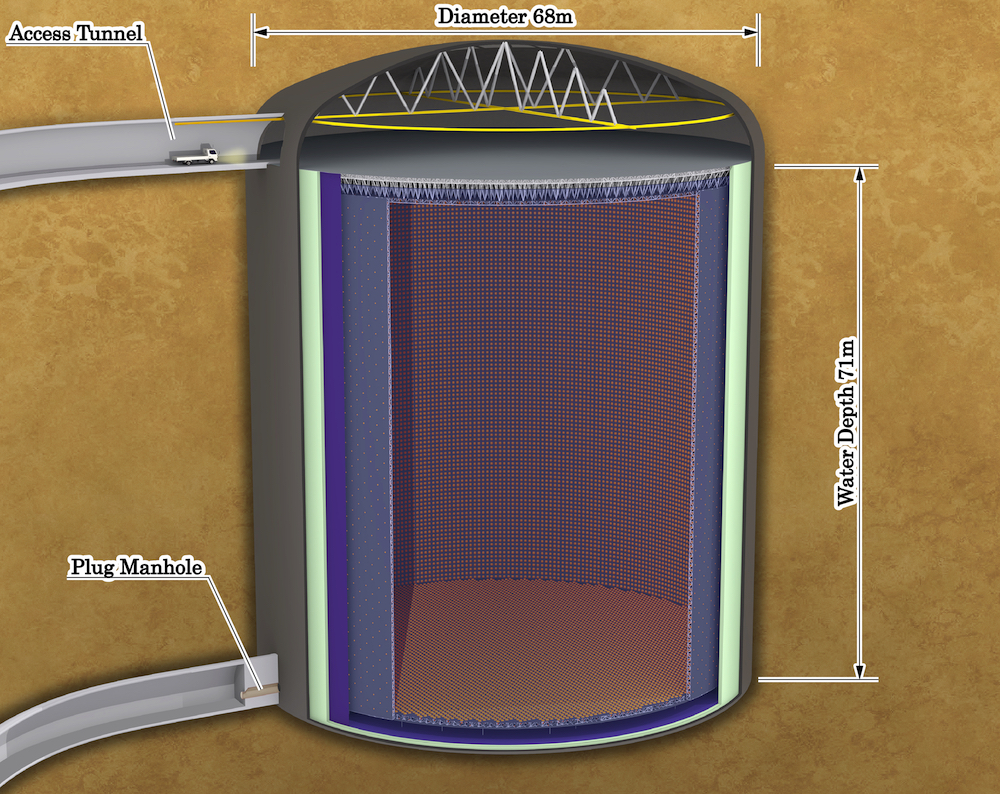